Gems Formed in Metamorphic Rocks
Over the eons, the interminable shifting of Earth’s landmasses has dramatically altered the appearance of our planet’s surface. The existence of ancient supercontinents such as Gondwana and Rodinia has fascinated the public since the concept of “plate tectonics” became widely accepted in the geological community in the 1960s. However, the gradual shifting of tectonic plates is of more than just “cosmetic” importance. The entire evolution of the Earth system is intricately related to tectonic activity, from the development of life on Earth to the creation of gemstone deposits. A previous installment of this column focused on gemstones produced through magmatic processes, which are associated with and controlled by tectonic processes. These tectonic forces also produce gemstone deposits by another process called metamorphism. In this process, gems form in the solid state in very specific situations when certain rocks experience an increase in pressure and temperature during tectonic events, with preexisting minerals no longer being stable and being replaced by new ones. This edition of Colored Stones Unearthed will focus on metamorphic gemstones.
INTRODUCTION TO METAMORPHISM
The term metamorphism derives from the Greek meta meaning “change” and morphos meaning “form.” The process of metamorphism describes changes in the mineralogical and/or structural form of rocks in the earth’s crust. Generally, this happens with increased temperature and/or pressure in the earth. Metamorphism is part of the so-called rock cycle involving igneous, sedimentary, and metamorphic rocks (figure 1). Any of these rock types can be transformed into one of the others by processes of melting and solidification (igneous), erosion and deposition (sedimentary), or increased pressure and temperature (metamorphic).
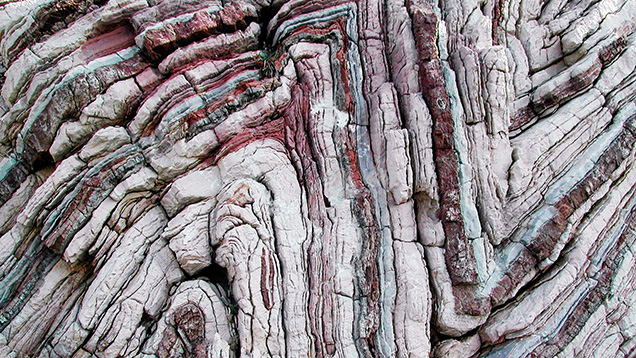
One of the more obvious ways in which metamorphism can be observed is in the physical appearance of a rock. At low pressure and temperature, rocks exposed to stress undergo brittle deformation, meaning they will break or fracture. However, at high pressure and temperature, exposure to directional stress in the earth can cause rocks to behave more plastically, even folding up into themselves, such as the limestone and chert layers in figure 2.
Another possible change during metamorphism is the alteration of a rock’s mineralogical composition. All minerals have specific sets of conditions at which they are stable. These conditions can include pressure, temperature, and chemical composition of the rocks in which they exist, as well as the presence or absence of coexisting fluids of various compositions. The naturally occurring mineral ice serves as a great example. At the earth’s surface, at sufficiently low temperatures, liquid water will freeze and form ice. As the temperature rises above 0°C, this ice will melt and form liquid water again. We can also alter the stability of ice by introducing other chemical components. As anyone living in a colder climate knows, adding salt to ice can lower its freezing temperature, making it easier for fresh snow or ice to melt, thereby minimizing slipping hazards.
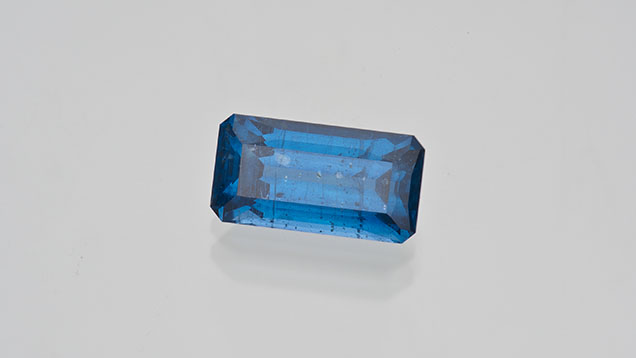
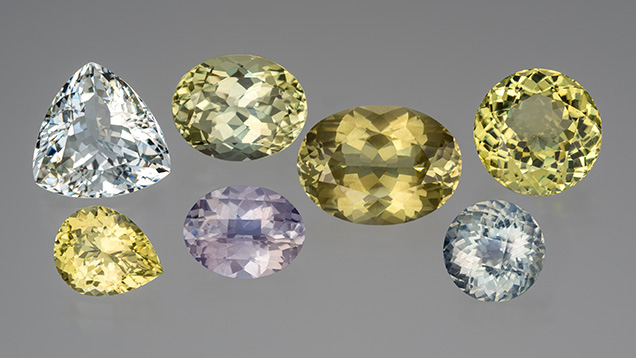
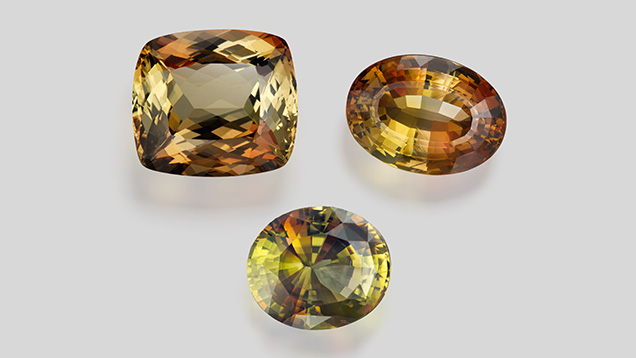
The same concept applies to every other mineral, and it is usually expressed through the use of phase diagrams. These phase diagrams plot the stability fields of certain minerals or mineral systems at various geological conditions. The most common phase diagram uses the variables pressure (P) and temperature (T). The phase Al2SiO5, which forms the gem minerals kyanite, sillimanite, and andalusite (figure 3), illustrates this concept. At conditions of very high pressure but lower temperature, the stable form of this phase is the mineral kyanite (figure 4). If a rock containing kyanite were heated up within the earth without changing the pressure, at a certain point it would cross over the boundary into the stability field of sillimanite (figure 5) and all the kyanite would be transformed in the solid state to this new phase (points 1 to 2 in figure 3). Similarly, if pressure were to decrease at this point without any drop in temperature, the sillimanite would transform to andalusite (figure 6) if the temperature were low enough as it crossed over to the new stability field (points 2 to 3 in figure 3). This diagram has helped metamorphic petrologists get a better sense of the metamorphic conditions experienced when one of these three mineral phases is encountered in a rock.
Of course, the earth is much more complicated than this simple Al2SiO5 system. In nature, rocks are made up of multiple components, and the minerals making up the rocks often are not pure phases but solid solutions between different end-member compositions. Therefore, the mineral assemblages observed depend not only on conditions of pressure and temperature but also on the composition of the original rock being metamorphosed (the protolith). Metamorphic petrologists use the concept of metamorphic facies to describe general ranges of metamorphic conditions at varying pressures and temperatures. Metamorphic facies are general ranges of pressure and temperature that produce a specific mineralogical assemblage that helps to identify those conditions at which a metamorphic rock formed. Figure 7 shows a diagram of these metamorphic facies from relatively low-grade prehnite-pumpellyite facies to high-grade granulite or eclogite conditions. Also shown on the top diagram are dashed lines roughly indicating the boundary at which dry or hydrated granite will start to melt. While partially melted low-grade migmatites may still be considered essentially the product of metamorphism, this represents the somewhat fuzzy boundary between metamorphism and igneous processes. The different metamorphic facies are generally distinguished by broad changes in mineral assemblages with changing metamorphic conditions. The expected mineral assemblages for each facies for a metamorphosed pelitic rock (known as a mudstone) are also shown in figure 7.
TYPES OF METAMORPHISM
There are multiple geological paths a rock can take toward metamorphism. The most straightforward is contact metamorphism, in which rocks are heated up due to nearby intrusion of a body of magma. Any changes to a rock’s mineralogical assemblage are due simply to an increase in temperature. It is assumed that there is essentially no exchange of chemical components between a protolith and the intruding magma or associated fluid. It is, then, an isochemical form of metamorphism.
The more important form of metamorphism for gemstone formation is regional metamorphism. In this case, rocks are buried deep within the earth and experience increased temperature and pressure as they become progressively buried. The most common scenario for this is during orogenic, or mountain-building, events when landmasses collide due to the shifting of Earth’s tectonic plates. The two most significant geological events leading to gemstone formation were the East African orogeny involving the collision of East and West Gondwana roughly 750–450 million years ago (Ma) and the Himalayan orogeny involving the collision of the Indian subcontinent with Asia starting about 60 Ma and continuing today. Gems from the East African orogeny are found across the eastern seaboard of the African continent as well as Sri Lanka, while gems from the Himalayan orogeny are located throughout Southeast and Central Asia in countries such as Vietnam, Myanmar, Pakistan, India, Afghanistan, and Tajikistan.
While scientists are fond of compartmentalizing nature into discrete classifications, these rock classifications typically have fuzzy boundaries and are not always clear-cut. For instance, classic metamorphism is considered to occur in the solid state without the melting of a rock or exchange of chemical components between different formations via fluids. However, this criterion is probably not strictly met, and most metamorphic reactions happen in the presence of some sort of fluid phase that may fundamentally alter the chemical composition of a metamorphic rock. Thus, the final form of metamorphism considered here is hydrothermal metamorphism in which changes in pressure and/or temperature are accompanied by changes in the chemical composition of the protolith by infiltration of hydrothermal fluids. This may also be described as “metasomatism” to emphasize the importance of both metamorphic and hydrothermal influences. For our discussion, this includes limestones or basalts that can be transformed into skarns or serpentinite, respectively, which in many cases may host gemstone deposits such as demantoid garnets.
METAMORPHIC GEMSTONES
Many of the world’s most important colored stone deposits are the product of metamorphism. Several of these deposits resulted from ancient to modern orogenic events when massive continental collisions buried rocks in the earth’s crust, fundamentally altering their mineralogical composition and in some cases producing fine gemstones. Sometimes hydrothermal fluids were an essential part of the process of gemstone formation in these metamorphic events. The following sections will provide a glimpse into the geological conditions of formation for some important metamorphic gemstones.
Jade. The term jade refers to two distinct gem materials that are characterized as extremely tough, essentially monomineralic fine-grained stones prized for their use in jewelry or ornamental carvings. The first type includes jade composed dominantly of the pyroxene mineral jadeite (NaAlSi2O6) but can also include the related minerals omphacite [(Ca,Na)(Mg,Fe2+,Al)Si2O6] or kosmochlor (NaCrSi2O6). This material is often referred to as fei cui. The second type is jade composed of amphibole minerals and is referred to as nephrite jade.
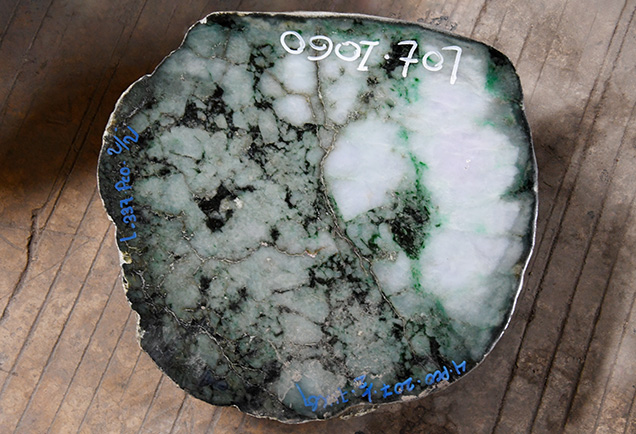
Fei cui jade is produced in subduction zone settings where convergent tectonic plate margins cause oceanic crust to be subducted deep within the earth. The subduction of relatively cold oceanic crust into the upper mantle creates a high-pressure, relatively low-temperature environment that is ideal for crystallizing jadeite. These conditions would be referred to as the blueschist up to eclogite metamorphic facies as seen in figure 7. Fei cui is essentially a rock and, as such, can record events in its geological history (figure 8). It occurs as veins or pods in bodies of serpentinized peridotites. These serpentinite bodies are the product of alteration of mafic or ultramafic igneous rocks by infiltrating fluids from the subducted oceanic crust in these geological settings. The same fluids responsible for serpentinization also likely played a role in the formation of jadeite. There is evidence that these jadeite bodies formed very deep within the earth, perhaps up to 80 km in depth, and were then exhumed or exposed later at the earth’s surface.
Nephrite jade is dominantly composed of amphibole minerals usually varying between the tremolite [Ca2Mg5Si8O22(OH)2] to ferro-actinolite [Ca2Fe5Si8O22(OH)2] end members. Nephrite jade is the product of hydrothermal metamorphism (or metasomatism) in which either (1) silica-rich fluids infiltrate dolomite-rich rocks, or (2) calcium-rich fluids from silicic rocks interact with serpentinites (Harlow et al., 2014). Metamorphic petrologists often describe the reactions implied above using reaction equations such as the following for the dolomite-related nephrite:
5 Ca(Mg,Fe)(CO3)2 + 8SiO2aq + H2O → Ca2(Mg,Fe)5Si8O22(OH)2 + 3CaCO3 + 7CO2aq
or more simply
5(dolomite) + 8(aqueous silica) + water → tremolite + 3(calcite) + 7(aqueous carbon dioxide)
These equations can help clarify the likely reactions that took place during metamorphic events and roughly track the metamorphic conditions required to facilitate these reactions.
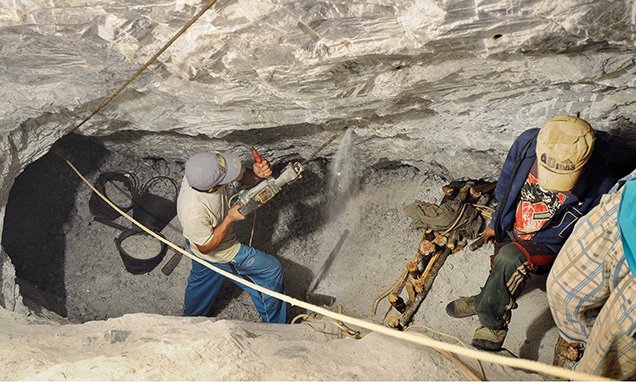

Ruby/Sapphire. Ruby and sapphire, gem varieties of the mineral corundum (Al2O3), can be broadly divided into metamorphic and magmatic varieties. The most significant deposits of metamorphic gem corundum were formed during two distinct geological events: the Pan African orogeny from 750 to 450 Ma and the Himalayan orogeny from 60 Ma to present. Sapphire from Sri Lanka, Madagascar, and Tanzania and ruby from Mozambique and Madagascar formed in the Pan African orogeny, while the Himalayan orogeny formed sapphire in Kashmir and Myanmar and ruby in Myanmar, Vietnam, Afghanistan, and Tajikistan. Metamorphic gem corundum forms in metamorphosed mafic or ultramafic rocks, marbles, gneisses, and metapelite complexes brought up to amphibolite or granulite facies conditions, generally at temperatures between 500° and 800°C (Giuliani and Groat, 2019). The rubies formed in the Himalayan orogeny at 620°–670°C at 2.6–3.3 kbar in marbles composed mostly of calcite (figures 9 and 10) within lenses of associated minerals including phlogopite, muscovite, scapolite, margarite, spinel, titanite, pyrite, and/or graphite (Garnier et al., 2008). Giuliani et al. (2015, 2018) also suggested the involvement of evaporate lenses within the original limestone protolith as a means of facilitating chemical exchange during metamorphism to form the rubies. In their model, it was suggested that molten evaporates may have infiltrated the carbonate layers, bringing along chemical components from clay layers and depositing corundum in veins and layers within the marble. Metamorphic sapphire deposits, such as those in Sri Lanka, Madagascar, Kashmir, and India/Pakistan, appear to require the involvement of metasomatic fluids infiltrating aluminum-rich protolith rocks during metamorphism to produce gem corundum (Atkinson and Kothavala, 1983; Silva and Siriwardena, 1988; Rakotondrazafy et al., 2008; Dharmaratne et al., 2012).
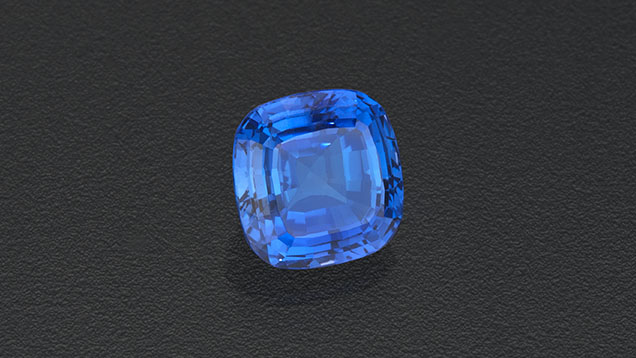
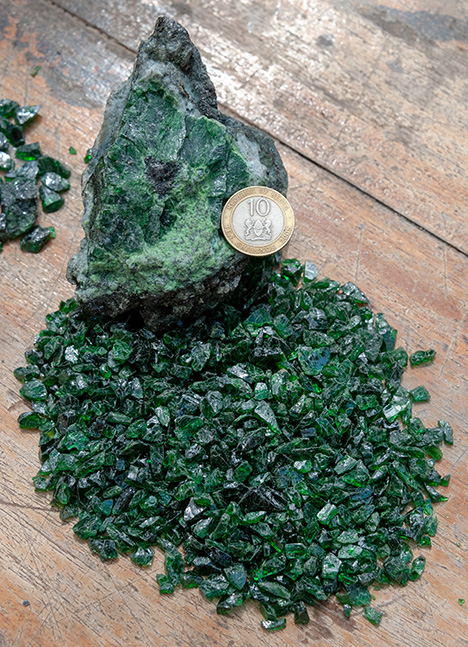
Tanzanite and Tsavorite. Tanzanite is the blue to violet variety of the mineral zoisite [Ca2Al3(SiO4)(Si2O7)O(OH)] (figure 11), while tsavorite is the green gem variety of the garnet mineral grossular (Ca3Al2Si3O12) (figure 12). Both were created in similar geological settings during the Pan African orogeny. The host rocks are a package of quartzite, graphitic gneiss and schist, calc-silicate rocks, and marble with lenses of meta-evaporites. The gems formed within nodules or lenses within these rocks. Tanzanite likely formed at around 420°C and 2–4 kbar of pressure (Giuliani et al., 2014), while metamorphic conditions in the formations containing tsavorite likely reached around 600°–750°C and 6–9.1 kbar (Malisa, 1987; Muhongo et al., 1999; Olivier, 2006). The original protoliths were sedimentary formations derived from continental weathering and later metamorphism during continental collision. Some studies have indicated the likely role of evaporate lenses through open hydrothermal circulation within these sedimentary packages for formation of these gemstones (Olivier, 2006) as suggested for the following reaction for grossular (tsavorite) formation (Giuliani et al., 2014):
3CaSO4 + 2Al3+ + 3SiO2 + 6H2O → Ca3Al2Si3O12 + 6O2 + 3H2S + 6H+
where CaSO4 is the mineral anhydrite and would have been sourced from evaporate lenses to create grossular garnet.
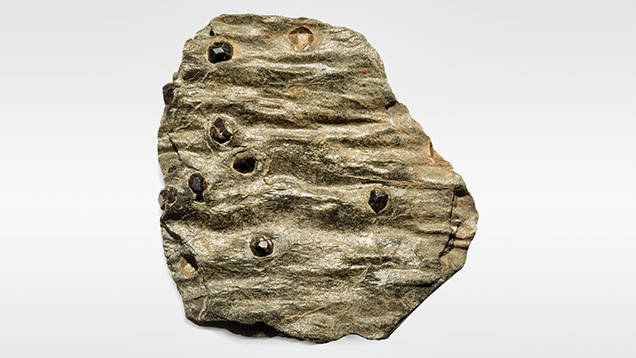

Garnet. As shown in figure 7, garnets (especially pyrope-almandine-spessartine type garnets [(Mg,Fe,Mn)3Al2Si3O12] tend to form only at conditions of increased pressure and temperature in metamorphic events. In fact, geology students learning metamorphic petrology will use the appearance of garnet in the field as a general indicator of the metamorphic grade of the host rock. Many of the red-colored pyrope-almandine-spessartine (pyralspite) garnets in the gem trade were derived from metamorphic rocks such as garnet schists (figure 13). Many more pyralspite garnets, including many color-change garnets (figure 14), are found in the Mozambique Orogenic Belt in East Africa and Sri Lanka. Their formation is assumed to be metamorphic in origin given the geological context, but they are generally found in secondary alluvial deposits. Therefore, their precise conditions of formation are typically not well understood because their original host rock is unknown and may have been completely removed by weathering.
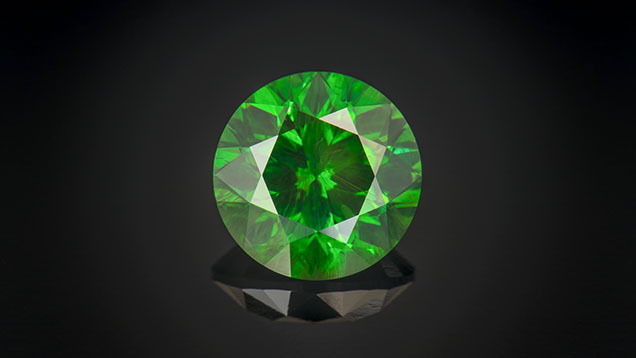
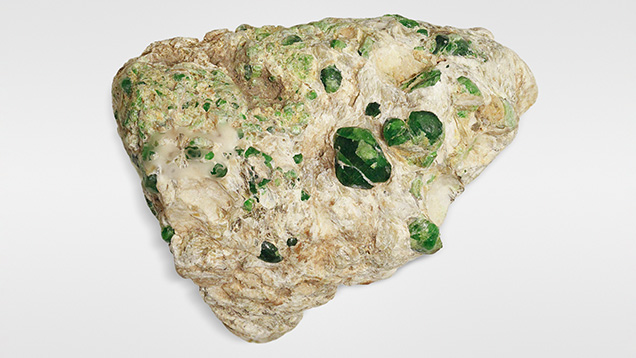
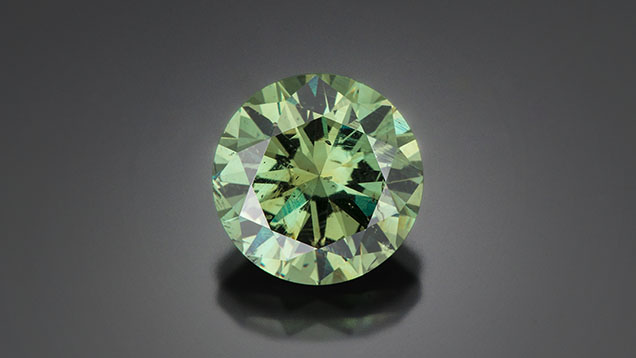
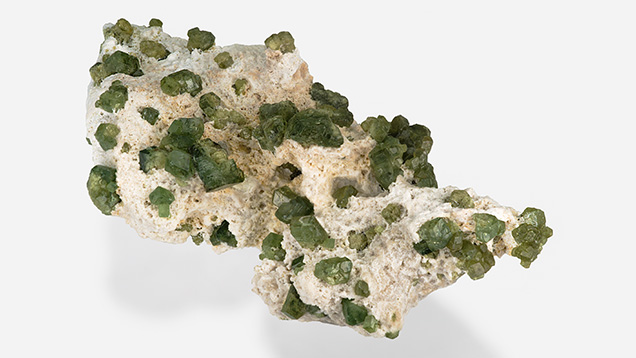
Most of these pyralspite garnets fit more squarely into the classical definition of solid-state metamorphism. But other gem garnets, such as demantoid, require more complicated metamorphic conditions involving not only increases in pressure and temperature but also the circulation of fluids to facilitate their formation. Demantoid is the green gem variety of the mineral andradite (Ca3Fe2Si3O12). These calcium-rich garnets form in two distinct geological environments. The first type of demantoid is found in serpentinized ultramafic rocks as in the classic deposit in the Russian Ural Mountains but also in Iran and Pakistan (Karampelas et al., 2007; Adamo et al., 2009). Here, demantoid forms in veins of serpentine mineralization cutting through peridotites and ultramafic rocks, facilitated by the circulation of hydrothermal fluids through these rocks. The second type is found in Madagascar and Namibia, and is related to skarn deposits in which the nearby intrusion of magma causes fluids to circulate through metasedimentary rocks rich in limestone or calc-silicate rocks (Pezzotta et al., 2011). Hand specimens of demantoid from both locales demonstrate the difference in geology through mineral associations: serpentinite-hosted demantoid occurs in a matrix of serpentine minerals (figures 15 and 16), while the skarn-hosted demantoid occurs in rocks dominantly composed of skarn minerals like diopside and wollastonite (figures 17 and 18).
SUMMARY
Many of the world’s premier gemstone deposits were derived from conditions of extreme stress, pressure, and temperature deep in the earth’s crust. These gemstones often bear signs of these metamorphic processes in their inclusions, which can mirror their geological conditions of formation. The study of these gemstones can offer insight into the earth’s history and the evolution of tectonic activity that led to their formation. The next installment of Colored Stones Unearthed will focus on the influence of sedimentary processes in the development of gemstone deposits.